All published articles of this journal are available on ScienceDirect.
Quantitative Assessments of the Liquefaction Hazard of Soils considering Possible Strong Earthquakes in Seismically Active Regions of Russia
Abstract
Introduction
The seismogenic liquefaction of the soil poses a great hazard to society and the environment. Therefore, it is actively studied in many countries. In Russian engineering and seismological practice, this area is not sufficiently developed. The deterministic approach still prevails in Russian research on this topic. More modern probabilistic estimates are very rare.
Methods
This paper describes examples of both deterministic and probabilistic assessments of the seismogenic liquefaction hazard performed in certain areas of the Russian territory with different seismogeological conditions. Deterministic estimates were made using the Iwasaki-Seed-Finn methods and their modifications. Probability distribution functions of a random variable, the “seismic potential of liquefaction” (SPL), were developed for probabilistic estimates. These functions are regional in nature and take into account two types of uncertainties. The first is the uncertainty in achieving “critical” values by the SPL value in the event of potentially dangerous earthquake sources for a given location. The second is the uncertainty in the very occurrence of these sources in a given place for a given period of time. The “critical” SPL values are determined by the strength properties of the site soils. All estimates are based on multivariate calculations using various models of strong ground motions and seismicity. In all cases, the probability of liquefaction of water-saturated sandy and sandy-loam deposits was estimated which were found near the seabed and at depths of up to 80 m in the waters of Pogibi cape (the coast of Sakhalin island), in the districts of Sochi and Novorossiysk, as well as in land conditions (Stavropol, Krasnodar).
Results
The results of the research made it possible to correctly (at the quantitative level) take into account this component of the seismic hazard of the studied territories.
Conclusion
The variants of practical use of the obtained data are offered. An assessment of the possibilities and limitations of the developed methodology is made, and ways to improve it are outlined.
1. INTRODUCTION
An analysis of the consequences of strong earthquakes shows that under intense seismic impacts, certain weak soils (water-saturated sandy, sandy-loam, sometimes loamy and gravelly) can experience thixotropic changes and behave like a viscous liquid. This phenomenon in engineering seismology is called seismogenic liquefaction. It is obvious that these processes pose a great danger to construction and other objects located in such seismic conditions. Therefore, the study of the nature of this event and the development of effective ways to predict it hold an important place in research on the engineering and seismological sphere. Such research has been particularly intensified in recent decades, which has led to significant progress in this sphere of knowledge. The achievements of the last decades in this sphere have been reflected in numerous publications, references to many of which can be found, for example, in the studies [1-14]. It is also noteworthy that the geography of such studies has expanded. If earlier they were largely concentrated in the scientific centres of the USA, Japan, Europe and China, now scientists from India, Pakistan, Iran, Ecuador, Turkey, and other countries are also actively working in this direction. Russian researchers have also made contributions, though the scale of such research in Russia has yet to reach the desired scale. The main issues considered by Russian researchers (see, for example [15-21],) usually relate to the identification of distribution areas of soils prone to liquefaction. Various characteristics of these soils are also considered in order to increase the accuracy of such definitions. It should be noted that many worldwide researchers, in principle, solve approximately the same tasks but with a larger-scale analysis of the geotechnical and seismological aspects of this problem. For example, when regional zoning of the territory is carried out according to the level of this type of seismic hazard (see [22-25]). A lot of work is dedicated to various methods of laboratory and field testing of different types of weak soils for their ability to liquefy and for the development of appropriate models (see, for example [26-31],).
In modern interpretation, forecasting the seismogenic liquefaction hazard of soil involves the definition of two main components. The first is an assessment of the strength properties of the soil, which determine its resistance to liquefaction under conditions of intense seismic influences. The second is to assess the possibility of such impacts occurring in a given area. At the moment, two approaches to solving the indicated problems are used in engineering and seismological practice – deterministic and probabilistic. Great progress has already been made in the development of deterministic assessments.
For example, various geotechnical characteristics affecting the liquefaction processes are considered with greater accuracy and detail, establishing a hierarchy of these characteristics and their interconnections.
Nevertheless, deterministic approaches have their limitations. Thus, a variety of natural factors that determine these types of seismic hazards, combined with incomplete knowledge about them, objectively create a mechanism of randomness. Failing to account for the uncertainties that arise in this case makes deterministic estimates not quite correct in some cases. Probabilistic approaches are more prospective here, as mentioned in a study [32]. Therefore, in recent years, the “probabilistic” direction has been intensively developing. However, in many cases, probabilistic estimates are limited only by considering the uncertainties associated with the reaction (liquefaction) of a potentially liquefiable soil. At the same time, seismic events capable of causing this reaction have been recognized. However, in reality, the occurrence of such a “recognized” seismic event also represents uncertainty, which should also be considered in the general formulation of the problem. For example, soils that are more prone to liquefaction and located in a less seismically active area may represent less hazard than soils that are less prone to liquefaction but located in a more seismically active zone. Therefore, this second type of uncertainty associated with the earthquakes themselves plays an important role and should be considered in the general probabilistic analysis of this type of seismic hazard of territories.
In Russian engineering and seismological practice, there are individual examples of the development and testing in specific seismogeological conditions of not only deterministic but also probabilistic approaches for assessing the seismogenic liquefaction hazard of weak soils. For example, studies of these types were previously carried out by the team of the North Caucasus Engineering and Geological Centre, “StavropolTISIZ” and “Izy`skatel`” companies, and recently by the Geophysical Institute of Vladikavkaz Scientific Centre of the Russian Academy of Sciences. However, as far as we know, similar studies in other regions of Russia have not yet received sufficient distribution. Therefore, at the moment, it seems useful to inform the interested audience about the research we have conducted in this direction and the results obtained, which is the main purpose of this paper.
2. MATERIALS AND METHODS
2.1. General Terms
Assessments of the seismogenic liquefaction hazard were carried out in relation to water-saturated sandy and sandy-loam layers widespread in the water area of Pogibi cape (the north-eastern coast of Sakhalin island) and in the water areas of Sochi and Novorossiysk cities (the Black Sea coast of the Caucasus), as well as on dry land in the area of Stavropol and Krasnodar cities (Central Caucasus). The research is based on the general “concept of critical statuses.” According to this concept, the liquefaction of weak (in our case, water-saturated sandy and sandy-loam) soils occurs after an increase in the pressure of the liquid in the pores of these soils above a certain (critical) level. The critical level depends on the strength properties of these soils. The reason for this increase in pressure is the vibration of the ground during earthquakes occurring in this area. Furthermore, to implement this concept, two types of initial data were used namely geotechnical and seismological. Geotechnical data, including engineering seismogeological sections, physical and mechanical characteristics of individual soil layers (including frontal resistance to probe penetration, density, degree of water saturation, granulometric composition, etc.), seismic wave propagation rates, absorption decrements were obtained from engineering and geological surveys conducted by specialized organizations at various times.. For the water area of Pogibi Cape, the following organizations contributed: the Institute of Marine Geology and Geophysics of the Far Eastern Branch of the Russian Academy of Sciences, the Far Eastern Marine Engineering and Geological Expedition, the Center for Regional Geophysical and Geoecological Research 'GEON,' Fugro Engineers B.V., and EQE International Inc. In the Caucasus region, contributions came from the North Caucasus Engineering and Geological Centre, the 'StavropolTISIZ' and 'Izy’skatel' companies, as well as the Geophysical Institute of the Vladikavkaz Scientific Centre of the Russian Academy of Sciences. Detailed information on the results of these studies is included in the reports kept at these organizations. The basis of initial seismological data is based on two groups of models developed first at the Institute of Marine Geology and Geophysics of the Far Eastern Branch of the Russian Academy of Sciences and then further improved at the Geophysical Institute of Vladikavkaz Scientific Centre of the Russian Academy of Sciences and the North Caucasus Federal University. Additionally, these models include strong motions for “average” soils and models of local seismicity. “Average” soils refer to the category II of soils based on seismic properties according to the Russian state construction standard Set of Rules 14.13330.2018, Seismic Building Design Code. The first group includes probabilistic attenuation functions with regional features include a distance to the source (D) of the average geometric values between the peak ground accelerations on two horizontal recording components (PGA), vibration durations at a level not lower than 0.5 PGA (t0.5), vibration periods with maximum amplitudes (TPGA) and Fourier vibration spectra |S|(f) during earthquakes of different potentially dangerous magnitudes (M). The second group includes models reflecting regional and local features of the zones of possible earthquake sources (PES zones), models of the repeatability of earthquakes of different M in time, models of the distribution of earthquake sources of different M in-depth, mechanisms of movement in the source, the size and orientation in the space of earthquake sources of different Magnitudes. A description of these models can be found in a study [33] and also in A. Yu. Chernov's PhD thesis, i.e., “Geoecological assessment of seismic hazard and risk on the example of urban areas of the Central Caucasus.” In this research, the assessment of the seismogenic liquefaction hazard is carried out in two types, deterministic and probabilistic.
2.2. Deterministic Estimates
In this case, both main indicators (both characteristics of soil resistance to liquefaction and parameters of seismic impacts) are considered as non-random values. This type of research was carried out for a section of the seabed in the water area of the Pogibi cape, as well as for one of the two land sites in the area of Stavropol. The determination of the “resistance” of the soil to liquefaction, in this case was carried out using the cyclic stress ratio (CSR), which is often used for these purposes. Estimates of the critical values of CSRcr (or in modern terminology CRR) were carried out using a well-known technique based on the empirical Iwasaki-Seed-Finn model [34-38] with further clarifications and additions [5, 6, 12]. As an indicator of the strength properties of soils, the data of the cone penetration test (СРТ) to determine the frontal resistance to penetration of the probe (qc) obtained in the process of engineering and geological surveys (see above) were used. When calculating CSRcr (or CRR), the lithological and granulometric composition of soils, the depth “on the ground” of the calculated point (h), and the depth of the sea (H) are considered. Based on the calculated CRR values, the values of the critical accelerations of ground vibrations (acr) derived from them are determined, at which the liquefaction of this type of soil occurs. The calculations of the acr were carried out taking into account the magnitudes of the earthquakes initiating the liquefaction. A detailed description of the calculation procedures, the selected parameters of seismic models, correction coefficients, and other details can be found in the aforesaid PhD thesis by A. Yu. Chernov. A deterministic assessment of potentially dangerous seismic impacts was carried out for specific scenarios of the most dangerous for the considered earthquake sites. At the same time, regional seismotectonic and local ground conditions are considered, as well as the depths of the calculated soil layers (h). At the same time, it is accepted that the degree of fragmentation of the Earth's crust, the mechanisms of movement in the source, and the depths and sizes of the source are close to those used in the construction of initial models of strong ground movements (SGM models). Therefore, amendments taking into account these factors were not introduced into the initial (basic) models. The soil conditions in the studied areas (which means “real” soils) differ from the “average” ones for which the basic SDG models are intended. Therefore, when making calculations, in accordance with Russian standards, it is accepted that when switching from “average” soils to “real” (III-IV category of soils by seismic properties according to the Russian state construction standard Set of rules 14.13330.2018 Seismic building design code), PGA values increase about 1.5 times over the entire range of changes in M and D. Attenuation functions TPGA(M,D) accepted the same for both “average” and “real” soils. During the transition from the surface to the internal horizons, “depth” (kh) corrections were introduced into the PGA estimates for the surface, considering the conversion of seismic signals (see [39]) and the change in soil properties with depth. Therefore, for example, in the area of the Pogibi cape at depths h = 0-10 m, soils of the III-IV category are deposited according to seismic properties at depths h = 35-45 m – II-III category, at depths h = 70-80 m – II category. Fig. (1) graphically shows examples of models used in further calculations of the frequency-dependent conversion coefficient kcon(h,f) and the resulting (total) correction “for depth” (coefficient kh(M,h)).
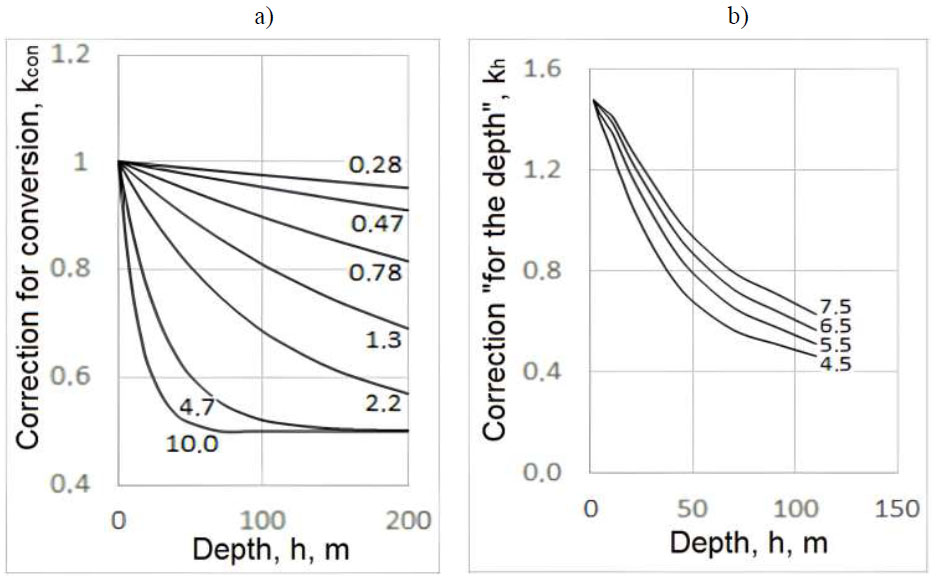
Examples of corrections used to recalculate the PGA values from the surface deep into the soil column: “a” – the values of kcon(h,f) accepted in the studies, where the numbers near the curves are the frequency in Hz; “b” – the resulting values of kh(M,h) accepted for the site in the water area of Pogibi cape, where the numbers near the curves are the magnitude of the earthquakes.
The values of M and D were taken according to PES zones. For assessments in all studied areas, PES zone models developed for the purposes of the general seismic zoning (GSZ) of the territory of Russia were used [40]. In addition, three more variants of PES zones were used for the area of Pogibi Cape including the model of the Institute of Marine Geology and Geophysics of the Far Eastern Branch of the Russian Academy of Sciences [41-43], the model of the Centre for Regional Geophysical and Geoecological Research “GEON” and model of the Far Eastern Marine Engineering and Geological Expedition. For the areas of Sochi, Novorossiysk, and Krasnodar cities, the PES zone models developed at the Sergeev Institute of Environmental Geoscience of the Russian Academy of Science were additionally used, and for Stavropol – from the Sсhmidt Institute of Physics of the Earth of the Russian Academy of Sciences. For each site, the final estimates were obtained by summarizing individual calculation results obtained using various options.
According to this approach, it is assumed that liquefaction occurs when the predicted values are as follows:
![]() |
(1) |
where PHA and PGA are in cm/s/s.
It is also accepted that water-saturated sandy and sandy loam soils with an index N1(60) ≤ 30 are potentially liquefied. At N1 (60) > 30, liquefaction is not possible at any level of seismic impact.
2.3. Probabilistic Estimates
The general approach to constructing the final probability distribution function of the considered random event (seismogenic liquefaction of soil) is similar to that described in a study [33, 44]. The final estimate considers both the conditional probability that when an earthquake occurs, the “liquefaction” parameter of seismic impacts will reach a critical level and the unconditional probability of the earthquakes occurrence. A mathematical description of the general calculation scheme is given in a study [44]. In this study, we only note that in this case, the final probability distribution function does not describe the occurrence of a random event i.e., soil liquefaction in the event of a “scenario” earthquake, but rather the integral (cumulative) probability of such liquefaction, taking into account all potentially dangerous earthquakes for a given place, with all possible M, and D as well as other characteristics, and taking into account the probability of occurrence of these earthquakes in space and time.
For probabilistic calculations, the same models of soil sections, local seismicity, and SGM models were used for deterministic estimates. The models t0.5(М,D) and |S|(М, D,f) were also used, which did not participate in deterministic calculations. The values of PGA, t0.5, and |S|(f) are considered random variables that have a lognormal distribution and standard deviations of ≈0.3 log units for all M, D, and f. The engineering and geological sections were used in a more generalized form. As with deterministic estimates, the initial models t0.5(М,D) and |S|(М,D,f) were corrected to take into account the transition from “average” to “real” soils, as well as the transition from estimates for the surface to estimates at different depths. At the same time, it has been assumed that on the surface, the values of t0.5 for soils of the III-IV category of the Russian Set of Rules in construction for seismic properties are on average two times higher than for basic “average” soils for all possible M and D. For soils with intermediate seismic properties, this coefficient was determined by interpolation. Fourier spectral densities for “real” soils are obtained by multiplying the initial values |S|(М,D,f) by a set of coefficients (φ(f) is a spectral characteristic showing the ratio between the spectra of “real” and “average” soils). Fig. (2) shows an example of the spectral characteristics of “real” soils for the seabed at a site in the area of the Pogibi Cape. The estimates were carried out using the method of thin-layered areas with absorption [39], considering additions arising from the recommendations of Russian standards and data on instrumental registration of felt and strong earthquakes [45]. The transition from estimates of spectra on the surface to estimates at internal points of soils is performed according to the same rules as for the PGA (М,D) models. Examples of such spectral characteristics for a site in the area of the Pogibi cape are also shown in Fig. (2).
As one of the models (“traditional”) for describing the parameters of strong motions initiating seismogenic liquefaction, multiplying PHA and t0,5 proposed in a study [35, 39] is used, which we will further call the “seismic potential of liquefaction” (SPL). In this research, the same M and D have been assumed:
![]() |
(2) |
where SPLtr in cm/s; PHA and PGA in cm/s/s; t0.5 in s.
The above-described PGA(М,D,h) and t0.5(М,D,h) models were used for SPL estimates.
The examples of SPLtr(М,D) models developed for the surface of “real” soils are shown in Fig. (3a). The same figure shows the parameters of |S|(М,D,TPGA) at frequencies corresponding to the values of TPGA(М,D). It can be observed from the figure that the curves |S|(М,D,TPGA) with a shift along the ordinate axis repeat the curves SPLtr(М,D). Fig. (3b) presents estimates of this shift, showing that the differences between the parameters |S|(М,D,TPGA) and SPLtr(М,D) are in a very tight range and practically independent of M and D. Based on this, the random variables |S|(М,D,TPGA) are also used as another option for estimating SPL, which we named “spectral” SPLsp(М,D).
The second important step is to select the level of the seismic potential of liquefaction (SPLcr), after which the process of soil liquefaction begins. In this paper, two such levels are defined – “optimal” and more careful (“conservative”). The first estimate is based on the parameter proposed in [39], equal to:
![]() |
(3) |
where Qcr in cm/s; PHA in cm/s/s; t0.5 in s.
We should note that this assessment is generalized and applies to the entire set of soils subject to liquefaction without considering the features included in this set of differences. Considering that:
![]() |
(4) |
where PHA and PGA are in cm/s/s.
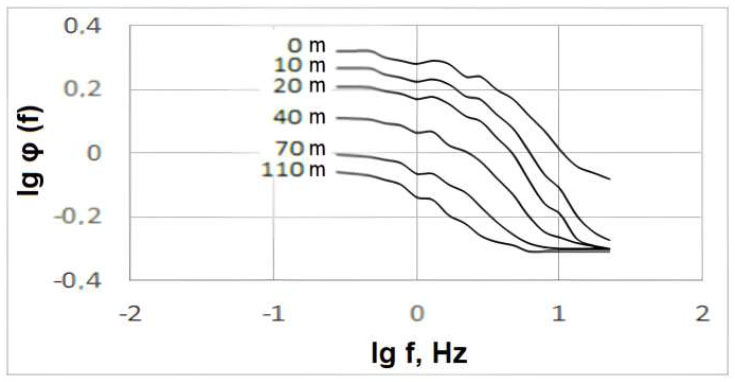
Spectral characteristics of “real” soils with ratio to “average” (φ(f)) at various depths h (numbers near the curves). The site in the water area of the Pogibi cape is shown in the Figure.
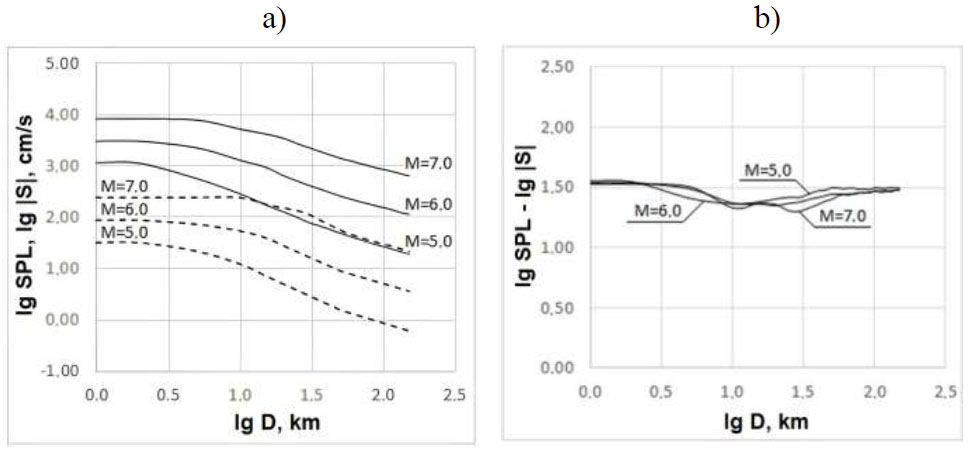
Examples of SGM models developed in this research to assess the possibility of seismogenic liquefaction of weak soils: “a” lgSPL at different M and D (solid lines) and lg|S| (dashed lines); “b” Deductions of lgSPL - lg|S| at different M and D.
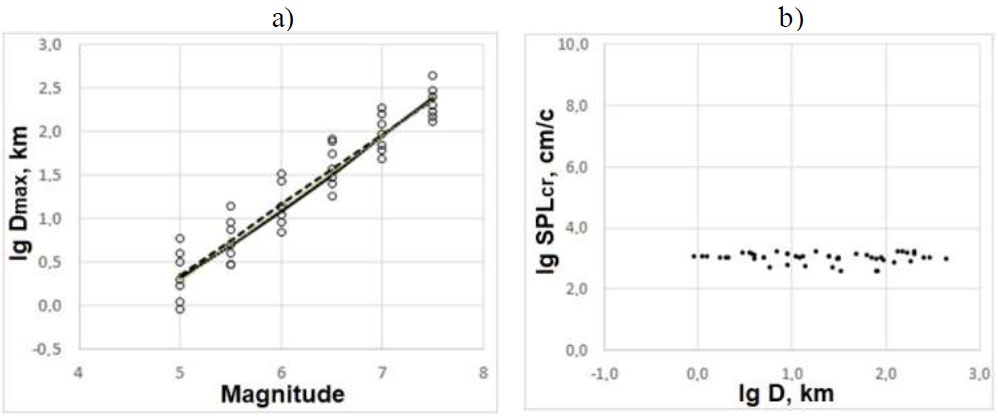
Examples of tests for the reality of SPLc estimates of this research: “a” Estimates of the maximum distances at which soil liquefaction was actually observed during earthquakes of different magnitudes (Dmax(М)), where circles and dashed line are data from different authors and their approximation, a solid line is estimated according to our models; “b” SPLcr parameters corresponding to estimates by different authors of the maximum distances Dmax (М).
In the “optimal” variant, the SPLcr parameter of this research is assumed to be 1 100 cm/s.
Furthermore, to verify the realism of our SPL estimates, tests were performed to ensure that they matched independent data. The results of one of them are shown in Fig. (4a). The high level of consistency of the data from a study [16-18, 46-48] is shown here on the limiting distances at which real cases of seismogenic liquefaction of soils were observed in earthquakes of different magnitudes and the same estimates resulting from our models SPL(М,D) and the “optimal” estimate of SPLcr. Fig. (4b) shows the results of another type of comparison of estimates using our SPL(M,D) models of the SPL parameters at the above-stated maximum distances of the really observed seismogenic liquefaction. The figure shows the stability of these SPL parameters at different M and D. The average lgSPL parameter is 3.03 or SPL=1 072 cm/s, which is very close to the “optimal” SPLcr parameter of the present research.
When determining the “conservative” estimate of SPLcr, the opinion of a number of researchers was considered (see, for example [39],), according to which, when taking into account the horizontal and vertical (PVA) components of soil vibrations, the SPLcr level may decrease. It can be supposed that in this case, the reduction coefficient may be equal to PMA/PGA, where:
![]() |
(5) |
where PMA, PGA and PVA in cm/s/s.
Based on the ratio PVA/PGA ≈ 0.70, we obtain PMA ≈ 0.82 PGA, and SPLcr ≈ 900 cm/s.
The above estimates – SPLcr = 1 100 cm/s and 900 cm/s are used in further calculations for all the studied areas on the seabed. Depth is considered by adding corrections for the coefficient of “elasticity reduction” (Rd) and “grain size.” An example of such corrections and final SPLcr(h) estimates for the area of the Pogibi cape is shown in Table 1.
The advantage of the described version of the approach is the possibility of correctly representing the SPL parameters in the form of probability distribution functions. The disadvantage is the large generalization of critical SPLcr values, which characterize some “average” soils in their ability to liquefy. The SPLcr values for different types of “real” soils may differ from the standard ones. Therefore, using the example of sites in the areas of Stavropol and Krasnodar cities tested another version of the estimates, in which all soils prone to liquefaction are divided into three groups which are “more favourable”, “average” and “less favourable”, characterized by values N1(60)>20, N1(60)=10-20 and N1(60)<10 accordingly. The above-defined base values of SPLcr=900 cm/s/s and 1 100 cm/s/s belong to the second group (“average”, N1(60)=10-20). For “more favourable” and “less favour- able” soils, the SPLcr values, respectively, increase and decrease by 20% compared to the base ones.
3. RESULTS AND DISCUSSION
In accordance with the described approach of deterministic definitions, calculations of effective (σ´) and total (σ) stresses, as well as pore pressure (u) for different depths in the soil (h), were performed. These data, together with the results of the СРТ –qc were recalculated into the number of SPT-impacts N1(60), for which two versions of CRR and aсr estimates were made (according to the criteria of W.D. Finn (Version 1) and according to the criteria of H.B. Seed, I.M. Idris, R.W. Boulange (Version 2)). In the area of the Pogibi cape, acr and PHA estimates were made for potentially dangerous earthquakes with M=5.0-7.5. The calculation results are graphically shown in Fig. (5a).
The results of such calculations for one of the two sites (with “more favourable” geotechnical conditions) in the area of Stavropol city (“Stavropol-1”) are shown in Fig. (5b). Fig. (5) shows that, according to deterministic estimates, seismogenic liquefaction of soils is possible up to depths of 22-25 m and 2-3 m, accordingly, at the site in the area of the Pogibi cape and at the site “Stavropol-1”.
In the area of the Pogibi cape, the distribution functions of the random value SPL are calculated in the depth range h=0-80m. At the same time, eight alternative distribution functions have been calculated for each of the depths, corresponding to different combinations of the used seismicity models (see above), “traditional” and “spectral” SPL models. The examples of estimates for exposition time te=50 years are shown in Fig. (6). Final estimates approximating these alternatives are also shown Fig. (6).
Based on the distribution functions of random values SPL and the boundary values of the SPLcr calculated for different depths from the surface (h), and estimates of the probabilities of non-occurrence of seismic events at a given location causing soil liquefaction were made. Graphically, the resulting functions obtained for the exposition time te=50 and 100 years at the site in the area of the Pogibi cape are shown in Fig. (7a). Fig. (7b) shows the results of similar calculations performed for the studied sites in the cities of Sochi and Novorossiysk.
h, m | Correction “elasticity reduction Rd” | Correction “grain size” | Total Correction | SPLcr | |||||
---|---|---|---|---|---|---|---|---|---|
(1/Rd)-1 | ΔSPLcr (cons.) | ΔSPLcr (opt.) | D50 | ΔSPLcr | ΔSPLcr (cons.) | ΔSPLcr (opt.) | Cons. | Opt. | |
0.0 | 0.000 | 0 | 0 | 0.35 | 0.00 | 0 | 0 | 900 | 1100 |
5.0 | 0.053 | 45 | 58 | 0.35 | 0.00 | 45 | 58 | 945 | 1158 |
10.0 | 0.111 | 94 | 122 | 0.35 | 0.00 | 94 | 122 | 994 | 1222 |
15.0 | 0.351 | 298 | 386 | 0.35 | 0.00 | 298 | 386 | 1198 | 1486 |
25.0 | 0.818 | 695 | 900 | 0.25 | 0.00 | 695 | 900 | 1595 | 2000 |
40.0 | 2.000 | 1700 | 2200 | 0.05 | 123 | 1823 | 2323 | 2723 | 3300 |
80.0 | 2.000 | 1700 | 2200 | 0.05 | 123 | 1823 | 2323 | 2723 | 3300 |
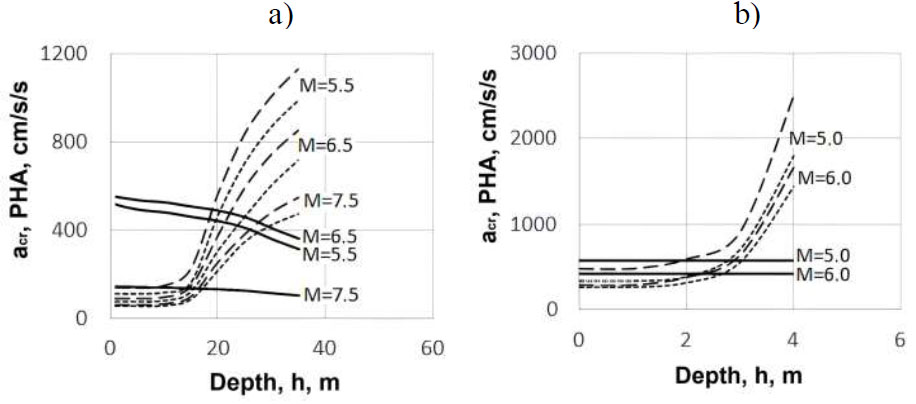
Deterministic assessments of the seismogenic liquefaction hazard of soil at different depths from the surface in earthquakes of different magnitudes (M) at sites in the areas of the Pogibi cape (“a”) and “Stavropol-1” (“b”). Dashed and dotted lines are estimates of aсr according to Versions 1 and 2; accordingly, solid lines are PHA estimates.
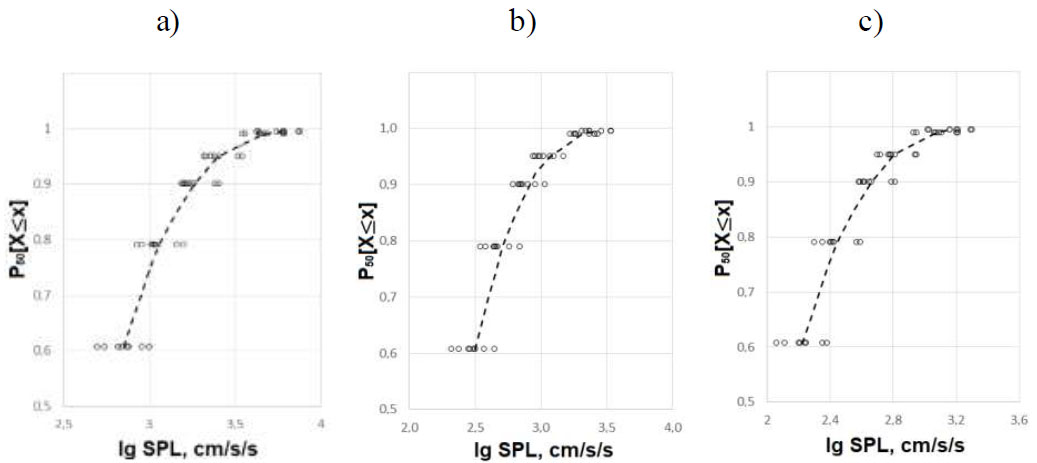
Examples of cumulative probability distribution functions SPL for different depths h. Exposition time is te=50 years. The water area of the Pogibi cape. Circles are individual estimates for different options, and dashed lines are approximate values. SPL in cm/s. “a” for h=0-12 m, “b” for h=25 m, “c” for h=80 m.
According to indicators N1(60), the soils at the “Stavropol-1” and “Stavropol-2” sites are “more favourable” and “less favourable” in terms of their tendency to liquefaction, and the soils at the site near Krasnodar city are “average”. Therefore, the probabilities of seismogenic liquefaction at the Stavropol sites were estimated according to the corrected values at the Krasnodar site according to the basic values (see above) of SPLcr(h). The final distribution functions for these sites are shown in Fig. (8).
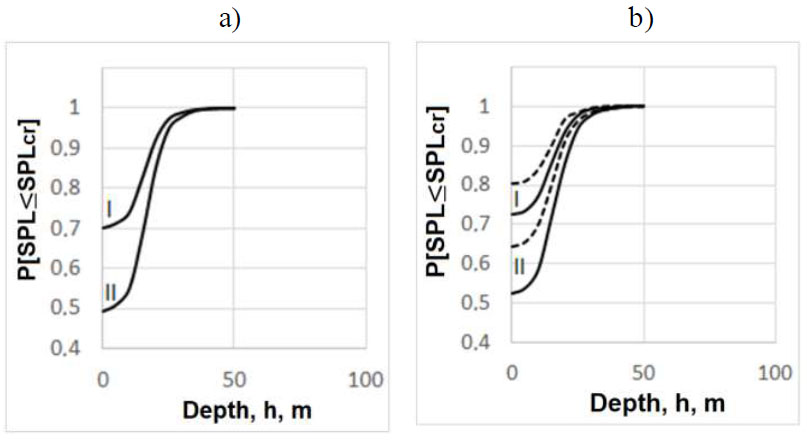
The probability of non-occurrence of seismogenic liquefaction of soil during exposition is te =50 years (I) and te =100 years (II) at various depths “on the ground” (h). “a” is the site in the area of the Pogibi cape, “b” presents the sites in the areas of Sochi (solid lines) and Novorossiysk (dashed lines) cities.
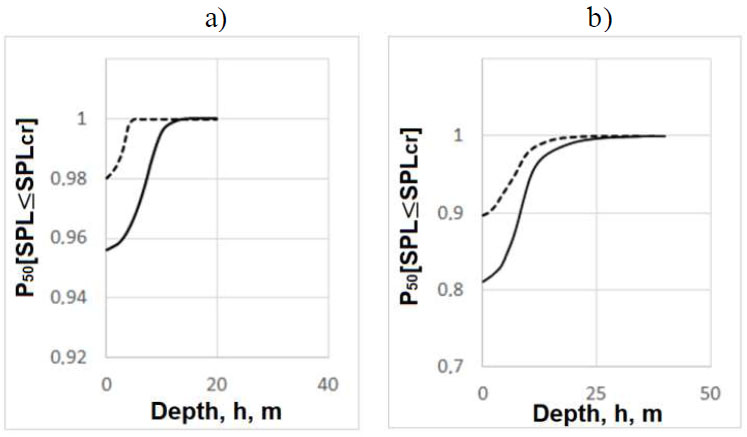
The probability of non-occurrence of seismogenic liquefaction of soil during exposition time te = 50 years at various depths “on the ground”. “a” – sites in the areas of “Stavropol-1” (dashed line) and “Stavropol-2” (solid line), “b” – site in the area of Krasnodar city, where the dashed and solid lines are “optimal” and “conservative” estimates accordingly.
h, m | ТSPL, years | |||||
---|---|---|---|---|---|---|
Area of the Pogibi Cape | Area of Sochi City | Area of Novoros-siysk City | “Stav-ropol-1” | “Stav-ropol-2” | Area of Krasnodar City | |
0 | 141 | 155 | 228 | 2 475 | 1 111 | 460 |
2 | 143 | 157 | 230 | 3 308 | 1 165 | 501 |
4 | 146 | 160 | 234 | 24 975 | 1 326 | 616 |
7 | 155 | 173 | 173 | ∞ | 2 248 | 975 |
10 | 167 | 193 | 288 | ∞ | 11 086 | 2 487 |
20 | 616 | 724 | 1 476 | ∞ | ∞ | 49 975 |
25 | 1 898 | 2 103 | 3 100 | ∞ | ∞ | ∞ |
40 | ∞ | ∞ | ∞ | ∞ | ∞ | ∞ |
Table 2 shows the average periods of repeatability of earthquakes accompanied by liquefaction (ТSPL), calculated (see [33, 44]) according to the final distribution functions shown in Figs. (7 and 8).
During the research, another type of assessment of the seismogenic liquefaction hazard was carried out, which we call conditionally probabilistic. The calculations were performed in several stages. First, the magnitudes of potentially dangerous earthquakes are determined for the calculated point (site). Then, through the distances for which the condition PHA(М,D)>acr(M) is true, the areas where liquefaction is possible in the event of earthquakes of these magnitudes are determined. Further, using the earthquake repeatability laws of different magnitudes related to this place, the frequencies of earthquake occurrence of the above (“modal”) magnitudes capable of causing (or rather exactly causing) liquefaction are calculated. The summation of the estimates of repeat- ability obtained for different magnitudes gives the total repeatability of seismic events leading to soil liquefaction in a given location - γcr and the average repeatability period Тcr=1/ γcr. The calculations carried out for the site in the area of the Pogibi cape have shown that, for example, at h =0, 2, 3, 10, 25, and 40 m, Тcr = 148, 150, 155, 165, 2 470 and >100 000 years accordingly. For the “Stavropol-1” site at h =0, 2, and 3 m, estimates of Тcr = 2 050, 2 560, and >100 000 years accordingly were obtained.
Comparing these estimates with the data in Table 2, we can observe their good convergence. Good mutual convergence is also observed, in general, between deterministic, probabilistic, and conditionally probabilistic definitions of the seismogenic liquefaction hazard of soil within all the studied sites. The results obtained do not contradict the generally available data in this field of knowledge. In addition, to a certain extent, this internal and external consistency can be regarded as evidence of the reality and reliability of the results obtained, which in turn gives reason to consider the described approaches workable.
When considering the practical use of this kind of information, it should be taken into account that the current Russian regulations do not directly indicate for which exposition time (design time period) probabilistic assessments of the liquefaction hazard for the research objects should be made. Therefore, for now, it seems it is necessary to focus on “individual” solutions. At the same time, it should be kept in mind that during soil foundation liquefaction, as a rule, damage is not possible in different degrees of severity (which is observed with vibration effects), but causes immediate destruction of the construction object. This, with all other factors being equal, leads to a relative increase in possible damage and, consequently, to an increase in the requirements for its prevention. In regular formats, for example, it is comfortable to do this by increasing the exposition time used in probabilistic hazard calculations. Therefore, by increasing the te period from 50 years were used in Russian estimates to 100 years and the probabilities of not exceeding 0.90, 0.95, 0.98 and 0.99 were used, which are usually considered “acceptable” for objects of different “degrees of responsibility,” we get “acceptable” repeat- ability periods for such objects ~ 1 000, 2 000, 5 000 and 10 000 years. Then, for example, in the areas in the water area of the Pogibi cape and Sochi city, the possibility of seismogenic liquefaction of soil for objects of “increased responsibility” with a probability of not exceeding 0.95 (since objects of “mass construction” with a probability of not exceeding 0.90 at the bottom of the sea are unlikely to be built) should be taken into account to depths of ~ 25 m, and in the water area of Novorossiysk city – up to ~ 20 m. In the same areas, for objects of “mass construction” (with a probability not exceeding 0.90) located on land, we obtain that the liquefaction hazard here should be considered to depths of ~ 22-23 m and to a depth of ~ 15 m in the area of Novorossiysk city. At the “Stavropol-1” site, the liquefaction’s hazard should be taken into account to a depth of ~ 2 m and only for “specially responsible” objects with a probability of not exceeding 0.98-0.99. At the “Stavropol-2” site, the liquefaction hazard is real to a depth of ~ 4-5 m for “mass construction” facilities and to a depth of ~ 7 m for “increased responsibility” facilities. In the area of Krasnodar city, such accounting is advisable for depths of ~ 7-8 m and ~ 10 m for “mass construction” and “increased responsibility” facilities accordingly.
The estimates given in this research are a first approximation. More accurate solutions can be obtained on the basis of probabilistic calculation and optimization on this basis of cost and other ratios between possible damages in the event of liquefaction and additional costs for preventing or reducing the probability of its occurrence due to the preparation of foundation soils – for example, by drainage, waterproofing, extraction of dangerous soil, its replacement or silicatization, the use of piles and other measures.
CONCLUSION
Overall, we can observe that probabilistic approaches to assessing the seismogenic liquefaction hazard of soil developed in combination with deterministic methods have shown good opportunities for effectively solving the problems that arise in this case. It is important to highlight that the estimates obtained do not relate to “scenario” earthquakes, the occurrence of which is predetermined in advance, but to earthquakes, the occurrence of which can be predicted only with some degree of uncertainty. Moreover, estimates are not made individually for one or more earthquakes, but the integral effect of the impact of a set of possible seismic events in a given place are estimated. Such estimates are funda- mentally different from “scenario estimates” because they are based on values that have different physical meanings.
The quality of the performed assessments ensures that the main natural factors determining the level of seismic hazard of these sites are taken into account, including the seismotectonic conditions of the areas and local engineering and geological conditions. The production of multivariate calculations also improves the quality of the estimates obtained. The final scores are verified through various cross-comparisons and correlations. The conducted studies have shown the operability of the proposed criteria for the seismic potential of liquefaction – SPLcr, as well as a “spectral” model of the seismic potential of liquefaction, which can be recommended for further use.
In addition to scientific and methodological value, the experience of this research has a certain practical value. Thus, the performed assessments showed that in all the territories under consideration, except for the “Stavropol- 1” site, the probability of seismogenic liquefaction of the upper layer of potentially liquefied soils is very high and should be considered when designing structures. In particular, this applies to objects of increased responsibility and to marine facilities.
Along with the positive aspects, it is necessary to notice the existing limitations. Therefore, the SPLcr criterion is still too generalized. Furthermore, to increase the accuracy of forecasts, it should be improved towards its differentiation in order to better account for variations in the engineering and geological properties of various types of potentially liquefied soils.
Separately, it should be noted that all estimates of this research relate only to “point-based” objects, the size of which is small. At the same time, the size of some structures (for example, pipelines, tunnels, bridges, dams, etc.) can significantly exceed the parameters of “point-based” objects. This requires special consideration of the seismic hazard for extended or “dispersed” objects.
Based on the above, the following conclusions can be highlighted:
1. The developed complex of probabilistic and deterministic approaches for assessing the seismogenic liquefaction hazard of soil has shown its effectiveness. The key element here is the ability to correctly and quantitatively consider not only the geotechnical characteristics of the considered soils but also the general seismogeological situation in which these soils are located.
2. The offered approaches make it possible to obtain the resulting information in the form of quantitative estimates of the probability of seismogenic liquefaction of the studied soil at a given location, which provides important guidelines for making optimal technical, economic, managerial and other decisions.
3. Geotechnical and engineering seismological models, which are the main components of the developed approaches, have sufficient flexibility and the ability to improve, which provides good prospects for further progress in this direction.
AUTHOR’S CONTRIBUTIONS
It is hereby acknowledged that all authors have accepted responsibility for the manuscript's content and consented to its submission. They have meticulously reviewed all results and unanimously approved the final version of the manuscript.
AVAILABILITY OF DATA AND MATERIALS
The data and supportive information are available within the article.